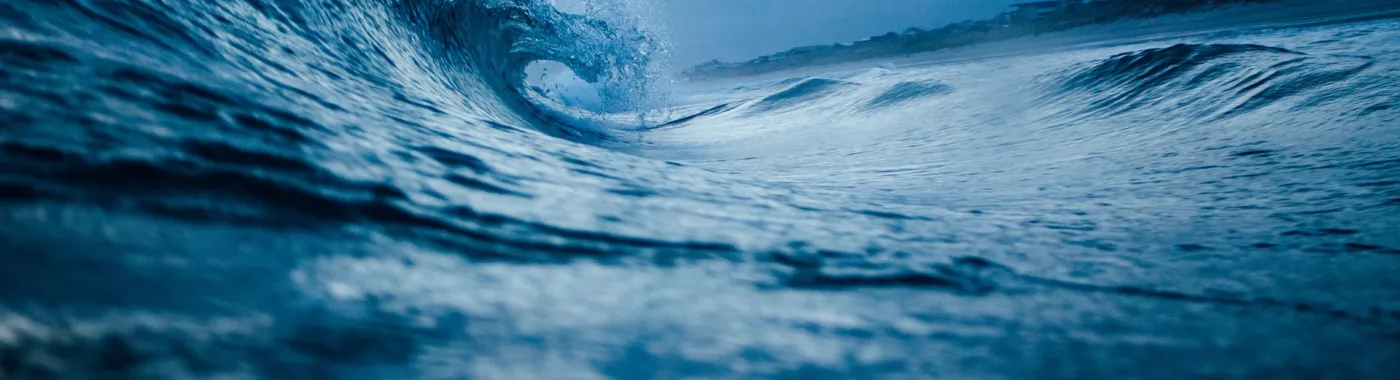
Currents, Waves, and Tides
Introduction
Looking toward the sea from land, it may appear that the ocean is a stagnant place. But this is far from the truth—the ocean is constantly in motion. Water is propelled around the globe in sweeping currents, waves transfer energy across entire ocean basins, and tides reliably flood and ebb every single day. But why does this occur?
Ocean movement is created by the governing principles of physics and chemistry. Friction, drag, and density all come into play when describing the nature of a wave, the movement of a current, or the ebb of a tide. Ocean motion is influenced by occurrences here on Earth that are familiar, like heat changes and wind. It also requires a shift in perspective to encompass the movement of planets, the Moon, and the Sun. Though it appears we live on a stable and stationary planet, we are, in fact, whipping through space around the Sun in an orbit and spinning on an axis. This planetary movement has a strong effect on how oceans move.
While the ocean as we know it has been in existence since the beginning of humanity, the familiar currents that help stabilize our climate may now be threatened. Climate change is altering the processes that propel water across the globe, and should this alter ocean currents, it would likely lead to a cascade of even more change.
Are You An Educator?
Currents
A large movement of water in one general direction is a current. Currents can be temporary or long-lasting. They can be near the surface or in the deep ocean. The strongest currents shape Earth’s global climate patterns (and even local weather conditions) by moving heat around the world.
Surface Currents
At the surface, currents are mainly driven by four factors—wind, the Sun’s radiation, gravity, and Earth’s rotation. All of these factors are interconnected. The Sun’s radiation creates prevailing wind patterns, which push ocean water to bunch in hills and valleys. Gravity pulls the water away from hills and toward valleys and Earth’s rotation steers the moving water.
Sun and Wind
Wind is a major force in propelling water across the globe in surface currents. When air moves across the ocean’s surface, it pulls the top layers of water with it through friction, the force of resistance between two touching materials moving over one another. Surface ocean currents are driven by consistent wind patterns that persist throughout time over the entire globe, such as the jet stream. These wind patterns (convection cells) are created by radiation from the Sun beating down on Earth and generating heat.
The Sun’s radiation is strongest at the equator and dissipates the closer you get to the poles. This uneven distribution of heat causes air to move. The hot air over the equator rises and moves away from the equator. Likewise, cold air from the poles sinks and moves towards the equator. The clashing of hot air originating at the equator and cold air originating at the poles creates regions of high atmospheric pressure and low atmospheric pressure along specific latitude lines. It would make intuitive sense that the hot air and cool air would meet in the middle of the equator and the North or South pole, however, in reality it is much more complicated. A combination of Earth’s rotation, the fact that Earth is tilted on an axis, and the placement of most continents in the Northern Hemisphere, create pressure systems that divide each hemisphere into three distinct wind patterns or circulation cells.
In the Northern Hemisphere, the most northern system, the polar cell, blows air in a consistent southwestern direction toward a pocket of low pressure along the 60-degree latitude line. The middle system, the Ferrel cell, blows in a consistent northeastern direction toward the same 60-degree low. And the most southern system, the Hadley cell, blows air in a consistent southwestern direction toward a region of low pressure along the equator. The result is a global pattern of prevailing wind, and it is this consistent wind that impacts the ocean.
While it may appear that the ocean is a flat surface, the reality is that it is a series of hills and valleys in the water. At the places where the wind generated currents converge into each other, the ocean water is pushed to build a slight hill. Likewise, where the winds diverge, the ocean water dips in a slight depression.
Gravity and Earth's Rotation
Wind pushes water into hills of high pressure which leave behind valleys of low pressure. Since water is a liquid that prefers to stay at a level height, this creates an unstable situation. Following the pull of gravity, ocean water moves from the built-up areas of high pressure down to the valleys of low pressure.
But as the water moves from hills to valleys, it does so in a curved trajectory, not a straight line. This curving is a result of Earth’s spin on its axis.
On Earth, movement in a straight line over long distances is harder than it may seem. That’s because Earth is constantly rotating, meaning every object on its surface is moving at the speed at which the Earth is spinning on its axis. From our perspective, stationary objects are just that, unmoving. In reality, they are whipping around at a speed of roughly 1,000 miles per hour (1600 km/hr) at Earth’s equator. It is that whipping, rotating motion that influences the movement of any object not in direct contact with the planet’s surface, making straight appearing trajectories actually bend. It also influences the movement of ocean currents. Scientists refer to this bending as the Coriolis Effect.
It is easiest to understand this phenomenon when thinking about travel in a northern or southern direction. Since Earth is essentially a sphere and it spins around an axis, anything near Earth’s equator will travel the fastest—since Earth is rotating at a constant rate and the equator runs along the widest part of the sphere, any object there must travel the entirety of Earth’s circumference in one rotation. As you get closer and closer to the poles, the distance traveled in one rotation gradually shrinks until it reaches zero at either pole. Therefore, an object on the surface will gradually spin slower the closer it gets to a pole.
But leave the surface of the planet, and the anchor keeping you in sync with the land beneath you disappears. Any moving object (plane, boat, hot air balloon, water) will begin its travels at the rotating speed of the location where it took off from. If it should travel north or south, the ground beneath it will be traveling at a different speed. Travel North from the Equator, and the ground will gradually spin slower beneath you. This causes an object attempting to travel in a straight line to veer to the right in the Northern Hemisphere and veer to the left in the Southern Hemisphere relative to the direction traveling.
Understanding how the rotating Earth affects movement to the west or east is a bit trickier. Envision an elastic string attached to a ball on one end and an anchored point at the other. The faster the ball is spun around the anchor, the more the elastic stretches and the farther the ball travels from the center point. An object traveling on Earth behaves the same way. If the object moves east, in the direction that Earth is spinning, it is now traveling around the axis of Earth faster than it was when it was anchored—and so, the object wants to move out and away from the axis. Still tethered by gravity, the object does so by moving toward the equator, the place on Earth that is the greatest distance from the axis. Travel west, the opposite direction that Earth is spinning, and now the object is spinning slower than Earth’s surface and so it wants to move toward the axis. It does so by moving toward the pole. This again appears as a bend to the right in the Northern hemisphere and to the left in the Southern hemisphere.
Water moving along Earth’s surface is also subject to the Coriolis effect which causes moving water to curve in the same directions described above. In the Northern Hemisphere, surface water curves to the right and in the Southern Hemisphere it curves to the left of the direction it is forced to move.
Swirling Gyres
Earth’s rotation is also responsible for the circular motion of ocean currents. There are 5 major gyres—expansive currents that span entire oceans—on Earth. There are gyres in the Northern Atlantic, the Southern Atlantic, the Northern Pacific, the Southern Pacific, and the Indian Ocean. Similar to surface waters, Northern gyres spin clockwise (to the right) while gyres in the south spin counterclockwise (to the left).
The center of the gyres are relatively calm areas of the ocean. The Sargasso Sea, known for its vast expanses of floating Sargassum seaweed, exists in the North Atlantic gyre and is the only sea without land boundaries. Today, gyres are also areas where marine plastic and debris congregate. The most famous one is known as the Great Pacific Garbage Patch, but all five gyres are centers of plastic accumulation.
Ekman Transport
Wind moving across the ocean moves the water beneath it, but not in the way you might expect. The Coriolis Effect, the apparent force created by the spinning of Earth on its axis, affects water movement, including movement instigated by wind. Recall that Coriolis causes the trajectory of a moving object to veer to the right or the left depending upon the hemisphere it is located in. But in this case, the three-dimensional nature of the ocean plays into the direction of the water’s overall movement. Wind blowing over water will move the ocean water underneath it in an average direction perpendicular to the direction the wind is traveling.
As wind blows over the surface layer of water, friction between the two pulls the water forward. As we know, when water (and other objects) moves across Earth’s surface it bends due to the Coriolis Effect. The top most layer of water will bend away from the direction of the wind at about 45 degrees. For simplicity, we will assume that this scenario is in the Northern Hemisphere and all movement bends to the right. As the top layer of water begins to travel, it in turn pulls on the water layer beneath it, just as the wind had. Now this second water layer begins to move, and it travels in a direction slightly to the right of the layer above it. This effect continues layer by layer as you move down from the surface, creating a spiral effect in the moving water.
In addition to a change in direction, each sequential layer down loses energy and moves at a slower speed. Friction causes the water to move, but drag resists that movement, so as we travel from the top layer to the next, some of the energy is lost. When all the layers down the spiral are accounted for, the net direction of the water is perpendicular to the direction of the wind.
Deep Currents
The ocean is connected by a massive circulatory current deep underwater. This planetary current pattern, called the global conveyor belt, slowly moves water around the world—taking 1,000 years to make a complete circuit. It is driven by changes in water temperature and salinity, a characteristic that has scientists refer to the current as an example of thermohaline circulation.
Both heat and salt contribute to the ocean water’s density. Saltier and colder water is heavier and denser than less salty (or fresher), warmer water. Around the globe there are areas where the heat and saltiness of ocean water (and therefore, its density) change. The most important of these areas is in the North Atlantic.
As warm Atlantic water from the Equator reaches the cold polar region in the North via the Gulf Stream, it rapidly cools. This region is also cold enough that the ocean water freezes, but only the water turns to ice. As the water freezes it leaves the salt behind, causing the surrounding water to become saltier and saltier. The cold, salty water then sinks in a mass movement to the deep ocean. It is this sinking that is a main driver for the entire deep-water circulation system that moves massive quantities of water around the globe. Cooling also occurs near Antarctica, but not to the extremes that happen in the Northern Hemisphere.
Another area of the ocean where massive amounts of water move to the ocean’s depths is in the Mediterranean. In this area, evaporation is the main driver that changes the salinity of the ocean water. As water in the Mediterranean evaporates, it leaves the salt behind. This super salty ocean water then bleeds into the Atlantic via the thin mouth of the Mediterranean, also known as the Strait of Gibraltar.
When cold, salty water circulates the globe and gradually becomes warmer, it begins to rise. The “old” deep water is full of nutrients that have accumulated from the sinking of waste from the productive surface waters up above. Locations where the “old” water rises are highly productive areas because they contain ample nutrients and have access to sunlight—the perfect combination for photosynthesis.
Currents and Change
Because ocean circulation is driven by temperature change, any variation to the planet’s climate could significantly alter the system. Scientists worry that the melting ice caused by global warming may weaken the global conveyer belt by adding extra fresh water in the Arctic. A 2018 study found that the massive ocean current that courses around the Atlantic Ocean, called the Atlantic Meridional Overturning Circulation, has decreased in strength by about 15 percent since 400 AD and is now the weakest it has been in 1,600 years. Ironically, despite an overall increase in global temperatures, many places in North America and Europe may get colder as a result.
Rip Currents
Not all currents occur at such a large scale. Individual beaches may have rip currents that are dangerous to swimmers. Rip currents are strong, narrow, seaward flows of water that extend from close to the shoreline to outside of the surf zone. They are found on almost any beach with breaking waves and act as “rivers of the sea,” moving sand, marine organisms, and other material offshore. Rip currents are formed when there are alongshore variations in wave breaking. In particular, rip currents tend to form in regions with less wave breaking sandwiched between regions of greater wave breaking. This can occur when there are gaps in sand bars nearshore, from structures like piers or jetties, or from natural variations in how waves are breaking.
Rip currents can move faster than an Olympic swimmer can swim, at speeds as fast as eight feet (2.4 meters) per second. At these speeds, a rip current can easily overpower a swimmer trying to return to shore. Instead of attempting to swim against the current, experts suggest not to fight it and to swim parallel to shore. For more safety tips visit NOAA’s guide to rip current safety.
Currents and Nature
Unseen by the human eye, thousands of microscopic animals hitch rides across oceans on an oceanic highway. These animals, called zooplankton, move at the whim of ocean currents. Off the Eastern Shore of the United States, one of the most powerful ocean currents—the Gulf Stream—is transporting zooplankton from the Gulf of Mexico, around the tip of Florida, up to Cape Cod in Massachusetts and then across the North Atlantic Ocean towards Europe. The currents enable the young creatures to find their way to hospitable places where they grow into adults.
Other ocean creatures hitch rides on currents using floating debris, like mats of seaweed, tree trunks, and even plastic. They use these havens to survive the otherwise perilous open ocean. After the 2011 tsunami that prompted the Fukushima Daiichi power plant meltdown in Japan, debris from the Japanese coast began washing ashore on the West coast of North America, bringing with it over 280 Japanese species. The movement of species across ocean basins helps maintain populations across the entirety of a species’ range. It also ensures the diversity of genetics within a population, an important factor for keeping species resistant and resilient to hardships like disease and environmental disasters.
Currents also influence where large adult species can and want to go. Turtles and whales migrate annually to the plentiful waters of Georges Bank off the coast of New England, a place that is productive because of the warm waters brought north from the equator.
Waves
Sculpting seawater into crested shapes, waves move energy from one area to another. Waves located on the ocean’s surface are commonly caused by wind transferring its energy to the water, and big waves, or swells, can travel over long distances.
When waves crash onshore they can make a significant impact to the landscape by shifting entire islands of sand and carving out rocky coastlines. Storm waves can even move boulders the size of cars above the high tide line, leaving a massive boulder hundreds of feet inland. Until recently, scientists attributed the placement of these rogue boulders to past tsunami damage, however, a 2018 study upended this notion by carefully recording the movement of boulders along a swath of rocky coastline in Ireland over a time period in which no tsunamis occurred. In addition to over 1,000 mid-sized boulders, many reaching over 100 tons in weight, scientists recorded the movement of a 620-ton boulder (the same weight as 90 full-sized African elephants), showing that storm waves moved it over 8 feet (2.5 meters) in just one winter.
Anatomy of a Wave
A wave forms in a series of crests and troughs. The crests are the peak heights of the wave and the troughs are the lowest valleys. A wave is described by its wavelength (or the distance between two sequential crests or two sequential troughs), the wave period (or the time it takes a wave to travel the wavelength), and the wave frequency (the number of wave crests that pass by a fixed location in a given amount of time). When a wave travels, it is passing through the water, but the water barely travels, rather it moves in a circular motion.
Wave Formation
Surface Waves
Waves on the ocean surface are usually formed by wind. When wind blows, it transfers the energy through friction. The faster the wind, the longer it blows, or the farther it can blow uninterrupted, the bigger the waves. Therefore, a wave's size depends on wind speed, wind duration, and the area over which the wind is blowing (the fetch). This variability leads to waves of all shapes and sizes. The smallest categories of waves are ripples, growing less than one foot (.3 m) high. The largest waves occur where there are big expanses of open water that wind can affect. Places famous for big waves include Waimea Bay in Hawaii, Jaws in Maui, Mavericks in California, Mullaghmore Head in Ireland, and Teahupoo in Tahiti. These large wave sites attract surfers, although occasionally, waves get just too big to surf. Some of the biggest waves are generated by storms like hurricanes. In 2004, Hurricane Ivan created waves that averaged around 60 feet (18 meters) high and the largest were almost 100 feet (30.5 meters) high. In 2019, hurricane Dorian also created a wave over 100 feet high in the northern Atlantic.
Giant waves don’t just occur near land. ‘Rogue waves,' which can form during storms, are especially big—there are reports of 112 foot (34 m) and 70 foot (21 m) rogue waves—and can be extremely unpredictable. To sailors, they look like walls of water. No one knows for sure what causes a rogue wave to appear, but some scientists think that they tend to form when different ocean swells reinforce one another. Many of the largest rogue waves recorded have been in the North Sea in the North Atlantic Ocean. One was recorded by a buoy in 2013 and measured 62.3 feet (19 m) and another nicknamed the Draupner wave was a massive wall of water 84 feet (25.6 m) high that crossed a natural gas platform on New Year's Eve, 1995.
Tsunami Waves
A classic tsunami wave occurs when the tectonic plates beneath the ocean slip during an earthquake. The physical shift of the plates force water up and above the average sea level by a few meters. This then gets transferred into horizontal energy across the ocean’s surface. From a single tectonic plate slip, waves radiate outwards in all directions moving away from the earthquake.
When a tsunami reaches shore, it begins to slow dramatically from contact with the bottom of the seafloor. As the leading part of the wave begins to slow, the remaining wave piles up behind it, causing the height of the wave to increase. Though tsunami waves are only a few feet to several meters high as they travel over the deep ocean, it is their speed and long wavelength that cause the change to dramatic heights when they are forced to slow at the shore.
Tsunami waves are capable of destroying seaside communities with wave heights that sometimes surpass around 66ft (20 m). Tsunamis have caused over 420,000 deaths since 1850—over 230,000 people were killed by the giant earthquake off Indonesia in 2004, and the damage caused to the Fukushima nuclear reactor in Japan by a tsunami in 2011 continues to wreak havoc. Although tsunamis cannot be predicted in advance when an earthquake occurs, tsunami warnings are broadcast and any waves can be tracked by a global network of buoys – this early warning system is essential because tsunamis can travel at over 400 miles per hour (644 km/hr). The highest tsunami wave reached about 1,720 ft (524 m), a product of a massive earthquake and rockslide. When the wave hit shore, it was said to destroy everything.
There are also other, usually less destructive tsunami waves caused by weather systems called meteotsunamis. These tsunami waves have similar characteristics to the classical earthquake driven tsunamis described above, however they are typically much smaller and focused along smaller regions of the oceans or even Great Lakes. Meteotsunamis are often caused by fast moving storm systems and have been measured in several cases at over 6 feet (2 meters) high. A 2019 study found that smaller meteotsunami waves strike the east coast of the U.S. more than twenty times a year!
Tides
Tides are actually waves, the biggest waves on the planet, and they cause the sea to rise and fall along the shore around the world. Tides exist thanks to the gravitational pull of the Moon and the Sun, but vary depending on where the Moon and Sun are in relation to the ocean as Earth rotates on its axis. The Moon, being so much closer to Earth, has more power to pull the tides than the Sun and therefore is the primary force creating the tides.
What Causes the Tides?
The Moon’s gravitational pull causes water to bulge on both the side of Earth closest to the Moon and on the opposite side of the planet. The Moon’s gravity has a stronger pull on the side of Earth that is closest to it, which makes the ocean bulge on that side, while on the opposite side of the planet the centrifugal force created by the Moon and Earth orbiting around one another pulls the ocean water out. Centrifugal force is the same force that smooshes riders to the outside walls of spinning carnival rides.
Meanwhile, Earth continues to spin. As Earth rotates, the water bulges stay in line with the Moon while the planet’s surface moves underneath it. A specific point on the planet will pass through both of the bulges and both of the valleys. When a specific place is in the location of a bulge it experiences a high tide. When a specific place is in the location of a valley it experiences a low tide. During one planetary rotation (or one day) a specific location will pass through both bulges and both valleys, and this is why we have two high tides and two low tides in a day. But, while Earth takes 24 hours to complete one rotation, it must then rotate an additional and 50 minutes to catch up with the orbiting Moon. This is why the time of high tide and the time of low tide change slightly every day.
The Sun also has a part to play in causing the tides, and its location in relation to the Moon alters the strength of the pull on the ocean. When the Sun and Moon are in line with one another they reinforce each other’s gravitational pulls and create larger-than-normal tides called spring tides. This happens when the Moon is either on the same side of Earth as the Sun or directly on the opposite side of Earth. Smaller-than-usual tidal ranges, called neap tides, occur when the gravitational force of the Sun is at a right angle to the pull from the Moon. The two forces of the Sun and Moon cancel each other out and create a neap tide.
Continental Interference
If Earth were a sphere covered by water, only the water would be able to move freely over the planet’s surface and the two tides in a day at each location would be more or less the same. But continents obstruct the flow of water, causing this seemingly simple daily cycle to be a bit more complicated. Because of continental obstruction, some locations experience two tides a day that are more or less the same height (known as semidiurnal tides), some locations experience one tide at one height and the second at a different height (mixed semidiurnal tides), and some locations have so much interference from land that they only experience one high tide and one low tide per day (diurnal tides).
The local geography can also affect the way the tides behave in a location. Shores around coastal islands and inlets may experience delayed tides compared to smoother surrounding coasts since the water must funnel in through constrained waterways.
Tides and Nature
The intertidal zone, the coastal area tides submerge for part of the day, is home to many ocean creatures. It takes a special set of adaptations to live a life half the time scorched by the Sun and the other submerged underwater. Moreover, the incoming tide promises a constant pounding by ocean waves. Despite this, it’s a place where species thrive. Shelled mollusks like periwinkles, muscles, and barnacles cling to rocks, sea stars wedge themselves in crevices, and crabs hide in fronds of algae.
Red Tide
A red tide is not a true tide at all but rather a term used to describe the red color of an algal bloom. Algae are integral to ocean systems, but when they are supplied with excessive amounts of nutrients they can explode in number and smother other organisms. The algae may produce toxins or they can die, decay, and the bacteria decomposing them take up all the oxygen. This massive growth of algae can become harmful to both the environment and humans, which is why scientists often refer to them as harmful algal blooms or HABs.
Monitoring Tides
Tidal movements are tracked using networks of nearshore water level gauges, and many countries provide real-time information with tidal listings and tidal charts. Tides can be tracked at specific locations in order to predict the height of a tide, i.e. when low and high tide will occur in the future. The Bay of Fundy in Nova Scotia, Canada has the highest tidal range of any place on the planet. The tides there range from 11 feet (3.5 m) to 53 feet (16 m) and cause erosion, creating massive cliffs. This erosion also releases nutrients into the water that help support marine life. The currents associated with the tides are called flood currents (incoming tide) and ebb currents (outgoing tide). Having reliable knowledge about the tides and tidal currents is important for navigating ships safely, and for engineering projects such as tidal and wave energy, as well as for planning trips to the seashore.
Additional Resources
Websites:
NOAA Tides and Currents
USGS Life of a Tsunami
UCAR Center for Science Education Thermohaline Circulation